This is not going to go well.
After 15 years of discussion and exploration…Twelve members of the International Subcommission on Quaternary Stratigraphy (SQS) voted against the proposal to create an Anthropocene epoch, while only four voted for it.
To be honest, this outcome has always been a strong possibility. There has always been a tension between the unquestionable fact that humans are now a geological force that is measurably altering our planet (the Anthropocene concept), and the debate over how you draw the arbitrary line in the geological record that codifies our rise to geological significance (the Anthropocene epoch). The long-standing contradictions that arise from using the same word as an evocative thematic header for the climate crisis, and also as a proposed addition to the precise and fussy terminology of chronostratigraphy, have now come to a head, and in the worst possible way1.
There has always been an undercurrent of resistance to this concept amongst the people who sit on the International Committee of Stratigraphy (ICS), of which the SQS is a part. The establishment of the Anthropocene Working Group in 2009 always felt a little grudging, the clear enthusiasm of some members like group chair Jan Zalasiewicz notwithstanding. And scientifically, I can understand some of their unease: trying to codify the effect of humanity on a geologic record that is still in the process of forming, on a planet that is still in the throes of responding to the giant poke we are giving it, does fly in the face of procedures and methodologies used to define boundaries deeper in geologic time: in a sense, we are trying to define the ‘golden spike’ for a still-moving target.
That said, I have long been extremely uncomfortable with the way this has been covered in the media, who have spent the last decade breathlessly covering ‘the search for the golden spike’, an approach which has completely blurred the line between the fact of the Anthropocene concept and the debate over defining its boundary. As I have argued in the past:
The worry was always that the media would treat the refusal to draw a line in the sediment that officially says ‘Anthropocene starts here’ as a rejection of the idea that humanity’s hands are now on the planetary steering wheel. And here we are:
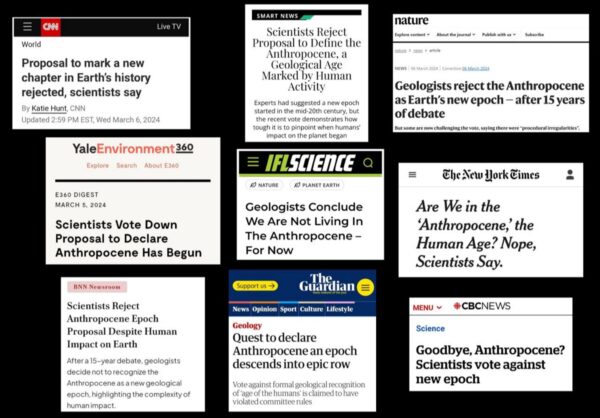
What happens now is important. The risk is that the SQS and ICS remain focussed on their internal debates, and don’t consider the impact of what they have decided on the current moment, where certain interests are willing to latch on any thing they can use to delay decarbonising our energy system. Geological history has been weaponised before (‘geology says Earth’s climate has always changed!’), and they will try to weaponise this, too.
So I dearly, dearly hope that they do not just look inwards, but reach outwards. They actually have a small window where they can grab the microphone and speak loudly and clearly that – golden spike or no golden spike – we are living in the Anthropocene2. It’s just too early to say exactly how the transition to the planet we are making will look in the rocks, tens of millions of years hence. But don’t doubt it: there’s going to be some really weird stuff for cockroach geologists of the far future to puzzle over.
- As the Nature article discusses, there is some noise being made about how the vote happened, but 4-12 is not exactly close – and even if it did somehow get wrangled over the hurdle presented by the SQS, there are still two more: a full vote by the ICS, and ratification by the International Union of Geological Science. And if you can’t win over the people who study the Quaternary – who have had their own battles with the ICS – I wouldn’t be optimistic about convincing people who think the Cretaceous is young.
- Even if they’d really, really prefer we not call it that. That ship has sailed.
Nice plan for content warnings on Mastodon and the Fediverse. Now you need a Mastodon/Fediverse button on this blog.